Introduction
Regulation of branching in plants, thanks to some new original concepts and intense experimental work, is now becoming better understood (Kebrom,2017). However, the integrity of a branching system in light of plant modular growth (Barlow,1989; Prusinkiewicz & Lindenmayer,1990) remains an open question and requires further investigations. There are many examples of branching patterns: branching shoots and roots or, on the tissue level, branching vascular strands. In seed plants, lateral shoots (branches) develop from axillary buds positioned below the shoot apical meristem (SAM), in the axils of leaves. Detached meristems located in these axils are discontinuous with SAM because of differentiation of intervening tissues. They have a potential to form axillary buds but may remain inactive (Evert,2007; Wardlaw,1965). In many gymnosperms, visible buds appear in the axils of but a few leaves among all initiated in one growing season (Fink,1984; Romberger et al.,1993). Moreover, especially in angiosperms, not all visibly formed buds become activated to elongate into lateral shoots. This process is regulated by plant hormones, mainly auxin, cytokinins, and strigolactones (Domagalska & Leyser,2011; Teichmann & Muhr,2015). Lately, the role of sugars produced by the leaves has also been shown to be important (Barbier at al.,2015; Fichtner et al.,2017; Mason et al.,2014). The process of bud activation must be under genetic control because the development of laterals leads to the species-specific shoot architecture (Bell,1991,1994; Hallé et al.,1978; Tomlinson,1983).
Every lateral is a new module of the branching shoot. It executes in the recursive way the same pattern of growth, organogenesis, and tissue differentiation as that of the supporting axis. Perpetuation of this pattern along the shoot vertical axis, during consecutive rounds of stem cells proliferation and their derivatives differentiation, is well described in terms of developmental stability of phyllotaxis or continuity of vascular system assured by homeogenic induction (Lang, 1965). In recent years, we have also gained some insight into genetic control of these processes (Biedroń & Banasiak,2018; Bhatia et al.,2018; Kierzkowski et al.,2013; Pinon et al.,2013; Scarpella,2017). Much less is known about the relationships between branches and their supporting axes. Are they totally independent modules? At least on the functional level, they must be integrated to assure continuity of transport and signaling. The development of their structural integrity on the levels of macro- and micromorphology is less clear. Possible coordination of their organogenesis and vascularization remains an open question. The literature pertaining to this problem is scarce. Quite early it has been recognized that in higher plants with alternate phyllotaxis the directions of leaf initiation (ontogenetic helices) within one branching unit composed of the parental shoot and its lateral branch may be correlated. Positive correlation is called homodromy, negative – antidromy (Dormer,1965; Raunkiaer,1919). The proportion of the two opposite correlations in a population of units varies among species (Wardlaw,1965) although antidromy is said to be more common (Dormer,1965). It suggests the presence of unknown control mechanism with variable, species specific effectiveness. Recently discovered, clear prevalence of antidromy in branching moss gametophores indicates that control mechanism is also present in lower plants (Zagórska-Marek et al.,2018). The specific tristichous phyllotaxis of moss gametophore is likely to create a horizontal gradient of putative signals, produced by the leaves and flowing vertically in the supporting (parental) axis. Recognition of the gradient by developing primordium of the lateral axis may drive it to become antidromous. The internal structure of moss axial organs is rather simple, thus it seems plausible to address the problem of branching shoot integrity using higher plant model such as coniferous shoot, in which not only superficial patterns but also the complex internal structures can be analyzed.
Biomechanics of a young, coniferous shoot with helical phyllotaxis in firs, spruces, or cedars largely depends on the presence of vascular sympodia and cortical resin canals. Vascular sympodia are composed of tracheary elements with thickened lignified cell walls and may be regarded as an inner skeleton of the shoot. Their strengthening role is intuitively obvious, while the role of resin canals was originally thought to be limited to the production of resin having antiseptic properties, thus representing plant defense system (Krokene & Nagy,2012; Moreira et al.,2015). However, quite rapid release of resin from the canals on the shoot transverse cut face proves that in the intact canals the resin is under high pressure. Contribution of resin canals to strengthening mechanically the coniferous shoot during its primary growth is evident. This conclusion is supported even further by the fact that these both intrinsic patterns with biomechanical properties are interlocked and related to helical phyllotaxis (Zagórska-Marek & Banasiak,2000). Vascular sympodia represent internal, vascular parastichies. They follow the course of one set of superficial helical lines connecting leaves (foliar parastichies) and thus are inclined to the shoot axis. The direction of this inclination, to the right or to the left, depends upon the chiral configuration of phyllotaxis (the direction of leaf initiation along ontogenetic helix) and anatomic diameter of the shoot (Buchholz,1938; Sinnott,1936,1960), which determines the number of sympodia in a vascular cylinder (Namboodiri & Beck,1968; Zagórska-Marek & Banasiak,2000). The number belongs to the mathematical phyllotactic series characterizing phyllotaxis of the shoot. In our earlier work, we found that inclination of cortical resin canals is always opposite to that of vascular sympodia (Zagórska-Marek & Banasiak,2000). The canals follow the course of opposite superficial parastichy set and their number is lower in a phyllotactic series. The development of such interlocked system is most likely due to heterogenic induction and may be treated as an example of correlation between two different patterns of cell differentiation.
The number of elements in the external cortical pattern, related but lower than that of the vascular bundles, makes resin canals within the cortex very conspicuous. They are more dispersed and distinct (discrete) than the bundles crowded in a concentric but smaller vascular ring (Figure 1). In addition, the ring accommodates, on a particular level, the departing leaf traces and their replacements (substitutes). The number of cortical resin canals is therefore a good marker of a true number of vascular sympodia in a particular shoot. How do these highly related systems of one shoot assemble within one branching unit composed of two modules – supporting, parental axis and the lateral one? Are their configurations independently established or related in any way that might suggest integration of developmental processes between the two axes?
Figure 1
Abies sp. – microscopic view of the shoot’s primary structure in a cross section. In the upper part of the photograph, the discrete cortical resin canals (crc) are visible; in the lower part, tightly packed vascular bundles (vb); their primary phloem cells are stained green. Scale bar: 100 μm.
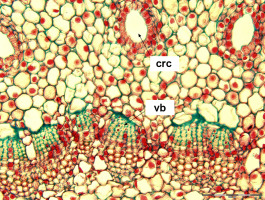
The systems described above allow answering these questions through studying branching shoot structure in detail. It appeared, and this is presented in the current paper, that coordination of developmental processes in branching units of conifers with helical phyllotaxis, as complex as they are, is amazingly precise! This case study also shows how careful one must be concluding on the presence or absence of this coordination.
Materials and Methods
We analyzed superficial patterns of leaf distribution and internal structures including systems of cortical resin canals and vascular sympodia in branching units using 2–3-year-old, highly branched shoots. Each of them consisted of many branching units. We defined single unit as an assemblage of one supporting and one lateral axis. The shoots were harvested in June and July from the terminal portions of big lateral branches of the old trees growing in the Botanical Garden of the University of Wrocław. The trees represented four taxa: Cedrus libani subsp. atlantica, Picea abies, Abies alba, and Abies sachalinensis (Figure 2).
Figure 2
Branching shoots of investigated coniferous species. Left photograph – Cedrus libani subsp. atlantica, middle – Picea abies, right – Abies alba. Scale bar: 30 mm, is the same for all photographs.
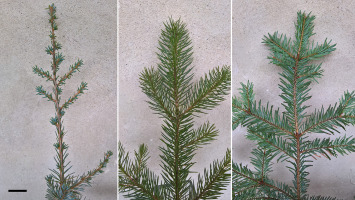
Phyllotaxis of each axis within the unit was recorded using contact parastichy pair formula (Figure 3A). First, we counted the numbers of helical lines connecting leaves (foliar parastichies) in two opposite sets. Next, we supplemented the numbers with S and Z indices of the lines orientation. From the formula, the chirality of ontogenetic helix (direction of leaf initiation) was deduced (Figure 3B).
Based on the number of cortical resin canals seen under stereomicroscope (SMZ745T; Nikon Instruments Europe, Poland) in the cross sections (Figure 3C), we determined and recorded the number of vascular sympodia and their chiral orientation for each shoot (Figure 3D). This simple protocol allowed screening quite a large population of shoot branching units in a relatively short time. Analysis of handmade fresh sections from randomly selected samples, photographed under Olympus BX50 fluorescence microscope equipped with an Olympus DP71 digital camera and CellˆB software, validated the above protocol of canals counting.
To elucidate the observed phenomena, we performed some in silico experiments using the latest version of Phyllotaxis software, written by Marcin Szpak (Zagórska-Marek & Szpak,2016). The software allows quantitative and qualitative analysis of developmental changes in phyllotactic patterns.
Figure 3
Protocol used to determine properties of phyllotaxis and vascular system. (A) Phyllotactic pattern identified through the formula of contact parastichy pair (blue underline). (B) Chiral orientation of ontogenetic helix deduced by extrapolating the empirically detected numbers of parastichies to the first number in phyllotactic series – in this case the main Fibonacci series. (C) The number of resin canals counted on the shoot cross section, based on the visible droplets of outflowing resin; information on canals chiral orientation drawn from extrapolated phyllotactic series. (D) The number and chiral orientation of vascular sympodia deduced from the number and orientation of resin canals.
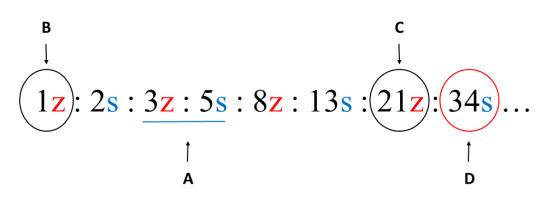
Results
Phyllotaxis – an External Pattern
Phyllotaxis in branching units of all investigated species was helical with clear prevalence of the main Fibonacci pattern. The numbers of foliar parastichies belonged in this case to the mathematical series 1, 1, 2, 3, 5, 8, 13, …. Expressions of this pattern, i.e., the opposed parastichy pairs determined on the basis of orthogonal system of most conspicuous parastichies (Adler,1974; Zagórska-Marek,1985,1994; Zagórska-Marek & Szpak,2008) varied depending upon the shoot diameter – were higher in thick leading shoots (e.g., 8:13, 5:8) and lower in smaller less vigorously growing ones (e.g., 3:5, 2:3). Other patterns were uncommon. Among them, as relatively frequent, we found main bijugy with parastichy numbers from the series 2, 4, 6, 10, 16, … and Lucas pattern with parastichy numbers 1, 3, 4, 7, 11, …. Other very rare phyllotactic patterns occurred but sporadically. Altogether, the patterns different than Fibonacci did not exceed 3.5%. This observation applied to all investigated species. The comparison of paternal axes chirality with that of the laterals revealed almost equal numbers of homodromic and antidromic cases in a population of branching units of Picea and Abies, suggesting random selection of lateral axis chirality (Table 1, Figure 4A,B – left scheme in each pair). In Cedrus, it was antidromy that clearly prevailed (Table 1, Figure 4C – left scheme).
Table 1
Frequency of chiral homodromic (H) and antidromic (A) correlations in studied plant material.
Figure 4
Paired schemes of phyllotactic and vascular correlations in the same branching shoot of Picea abies (A), Abies alba (B), and Cedrus libani subsp. atlantica (C,D). The orientation of ontogenetic helix (the chirality of phyllotaxis) and vascular parastichies is to the left (blue) or to the right (red). Black dot marks the border between two consecutive annual growth increments. Number and percentage of homodromous (H) and antidromous (A) correlations are given below each scheme. In (A), phyllotactic correlations (left scheme) suggest random selection of the lateral shoot chirality; vascular correlations (right scheme) show that orientation of vascular sympodia is uniform: to the left in the main axis and the same in all branches of this shoot. Also in (B), phyllotactic correlations suggest random selection of the lateral shoot chirality, whereas vascular correlations show that orientation of vascular sympodia is uniform in each branching unit: in the main axis initially to the right and the same in all branches; reorientation of vascular sympodia in the main axis to the left is followed by the same orientation of vascular system in the three subsequent laterals. In (C), phyllotactic antidromous correlations suggest nonrandom selection of the lateral shoot chirality; vascular correlations show that orientation of vascular sympodia in the laterals is adapting to ever changing situation in the main axis in order to maintain homodromic correlation. (D) is the shoot, in which branching took place also in the lower part of annual increment; phyllotactic correlations suggest random selection of the lateral shoot chirality; vascular correlations show that with only one exception, orientation of vascular sympodia in the laterals is adapting to changing situation in the main axis in order to maintain homodromic correlation.
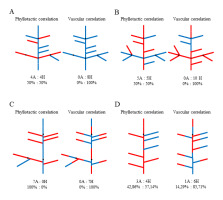
Internal Systems
The number of cortical resin canals was determined for each axis below and above the level of lateral branch departure. The number sometimes changed ontogenetically along the length of one annual increment of the shoot. The change was unpredictable in the case of Picea and Abies shoots. In Cedrus, however, the number changed regularly during one growing season, usually from 13 to eight canals in the shoots with Fibonacci phyllotaxis (Figure 5). Branching in Cedrus was observed mainly in the upper part of the annual shoot increment.
Figure 5
Two transverse handmade sections through the annual increment of the Cedrus shoot with Fibonacci phyllotaxis. On the left – bottom part of this shoot with 13 cortical resin canals (crc), on the right – the upper part with the number of canals reduced to eight. The change annunciates the vascular system (vs) reorientation. Scale bar: 500 μm.
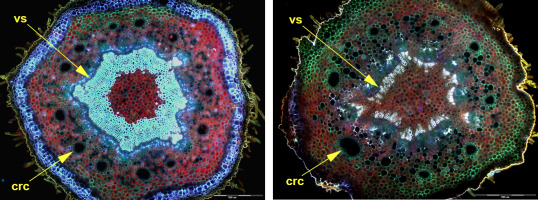
Based on the number of cortical resin canals combined with information on phyllotaxis expression and chirality, we reconstructed the intrinsic architecture of each shoot paying special attention to the orientation of elements making up interlocked system. In the second step, we compared both shoots composing a branching unit. In all cases, including the special case of Cedrus, vascular homodromy was clearly prevailing, approximating 90% on average (Figure 4 – right scheme in each pair, Table 1). This strong correlation applied also to the units in which phyllotaxis different than Fibonacci: Lucas or bijugy, was present in either of the two shoots.
This result contradicted the conclusions drawn from the previous analysis of phyllotactic correlations. We also noted that when, in the main supporting axis, the number and orientation of vascular sympodia changed ontogenetically, it was immediately followed by the adaptive change in orientation of vascular sympodia in all subsequent laterals (Figure 4B–D – right schemes in each pair).
It was especially obvious in Cedrus where the sympodia number and thus their orientation in the supporting axis were changing regularly within one growing season. Continuation of vascular homodromy in branching units despite the change in the supporting axis indicated the importance of this correlation and existence of unknown mechanism controlling the integrity of the whole vascular system in a branching shoot.
In Silico Experiments
To understand the empirically detected axial ontogenetic changes in the number of vascular sympodia resulting in their wavy course, we performed some in silico experiments in which the size of leaf primordia relative to the size of shoot apical meristem’s organogenic surface was changing systematically. Their results showed that the continuous change, at some well-defined and discrete moments, resulted in a sudden rearrangement of connection between the leaves. It happened always when the shorter routes of connections became available (Figure 6).
Figure 6
A computer simulation showing the effects of continuous increase in leaf primordia size (red circles) initiated within simulation space of constant width, which represents the size of shoot apical meristem’s organogenic zone (split open). White fine lines are the opposite parastichies connecting leaves along the shortest routes. The leaf traces make up the vascular sympodia (vascular parastichies) under two imperatives: to be the shortest and as vertical as possible. The initial 13 sympodia change into eight. In the lower part of the simulation, the connections between every eighth leaf are more horizontal than between every thirteenth leaf. In the upper part, suddenly the connections between every eighth leaf become shorter than between every thirteenth leaf. From that moment on, the number and orientation of sympodia change. The change in their orientation is marked with yellow line. Interface between the two domains of opposite sympodia orientation is serrate.
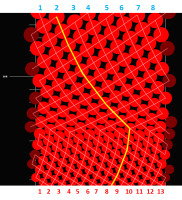
Discussion
In conifers, SAMs in principle do not change the direction of leaf initiation (chirality of phyllotaxis) between years, despite periodically changing organ identity from the leaf proper to cataphylls and vice versa (Zagórska-Marek,1985). One significant exception from this rule is Torreya case, where every year the direction of leaf initiation is selected at random (Banasiak & Zagórska-Marek,2006). The difference between Torreya and other surveyed genera such as Picea or Abies is in the presence or absence of vascular strands in the vicinity of SAMs organogenic zone. This finding validates the role of vascular system in regulating SAM’s organogenic activity postulated by Larson (1975). The experiments performed with use of pin1 mutants of a model plant Arabidopsis (Banasiak,2011; Banasiak et al.,2019) confirm this role. In this work, we go further by demonstrating that function of the lateral SAM in a branching unit of coniferous shoot is affected by the condition of vascular system in the supporting axis.
The beginning of leaf primordia initiation in the axillary bud of conifers is preceded by formation of two opposite prophylls (Evert,2007). In light of this neutral, achiral condition resembling early development of embryonic shoot in dicots it was plausible to assume that the chirality of the lateral branch phyllotaxis is selected at random and is not affected by the supporting axis. Our finding of obligatory vascular homodromy in branching units falsifies this hypothesis. Concordant chirality of vascular system in branching units indicates that the direction of leaf initiation (ontogenetic helix) in a lateral bud must be adjusted to the condition existing in the parental axis. The orientation of sympodia in the lateral will be the same when its anatomic diameter and thus sympodia number is identical or significantly different, i.e., much smaller or much bigger than in the supporting axis. In this case, phyllotaxis of the lateral is homodromous. Phyllotaxis of the lateral with slightly smaller or bigger diameter must be antidromous – only this guarantees the same orientation of vascular sympodia. Let us analyze in detail the case of branching shoot with the main Fibonacci pattern shown in Figure 4. Assuming that in the main axis with S phyllotaxis there are 21 sympodia oriented to the left (S) it must be concluded that in the phyllotactically homodromous laterals the number of sympodia is either the same – 21 or, in the case of much weaker shoots – eight. In the case of antidromous laterals, their number of vascular sympodia should be 13. In the typical situation observed in Cedrus, exemplified by the scheme shown in Figure 4D, after developmental change of vascular sympodia number in the main axis from 21 (as indicated by 13 resin canals) to 13 (with eight resin canals), the subsequent laterals must be phyllotactically antidromous and have anatomic diameter increased in order to have sympodia number 21. Laterals developing in the lower portion of the shoot’s annual increment should always be homodromous to have the same number and orientation of vascular sympodia as the parental axis. Because branching in this area is less frequent, we come to a very nice explanation of phyllotactic antidromy prevalence in Cedrus. Interestingly, vascular homodromy is totally independent of the quality of phyllotactic pattern present in both components of one branching unit – it was also observed when either parental axis or the lateral had a pattern other than main Fibonacci – Lucas or bijugy.
The importance and the leading role of the vascular system in regulating organogenic activity of the shoot apical meristem, evidenced by obligatory vascular homodromy in conifers, is especially strengthened by the fact that this correlation is maintained within one growing season even when the number and inclination of vascular sympodia alters without changing quality of the superficial pattern of leaf distribution. This situation regularly takes place in Cedrus (Figure 4C,D, Figure 5) but also, sporadically, in other species investigated in this study (Figure 4B). Similar ontogenetic changes were described in angiosperms. Puławska (1965) reported that they occur regularly in the ontogeny of Actinidia shoots. Sudden change in the vascular architecture during continuous, quantitative changes in phyllotaxis may be perversely assessed as an example of the Hegelian quantity-to-quality transition (Kolb,2005). Why the change is so sharp explains closer analysis of phyllotactic changes in computer simulation (Figure 6). When the consecutive leaf primordia sizes change continuously relative to the size of organogenic space, the existing vascular parastiches also change in their inclination to the vertical axis (Zagórska-Marek & Szpak,2008). The length of leaf traces making up a particular vascular parastichy increases as well as the energy cost for their differentiation. Last but not least, the route of future transport elongates. Then, the moment comes when the shorter route becomes available through more vertical vascular connections, along the opposite parastichy, and this initiates rearrangement of leaf traces. As a result, the new number of oppositely inclined vascular sympodia emerges assuring both, most vertical and most economic transport along the shortest route. The computer simulation (Figure 6) also shows that the interface of two domains, which have opposite orientation of vascular strands, is not exactly horizontal – this might explain a small fraction of cases in which the principle of vascular homodromy is not observed (Table 1, Figure 4D).
The discovery of vascular system changing orientation in the shoot ontogeny has far reaching implications for the further development of cambium during the shoot secondary growth. We propose that this may be one of the factors triggering the periodic changes in cambial cell orientation leading to the formation of dynamic wavy patterns in cambium and secondary xylem (Hejnowicz,1974; Zagórska-Marek,1995; Zagórska-Marek & Hejnowicz,1980).